The neutrino is a particle that almost isn’t here. It has no charge, almost no mass, and not even a fixed identity: it comes in three subtly different forms, and any given particle constantly shifts between them. About 500 trillion neutrinos fly into your body every second, and then they fly right back out without a trace. Neutrinos treat the rest of the Universe with indifference. ‘If you sent a neutrino at a lead wall that was a light-year thick, there’s a 50 per cent chance that neutrino would go all the way through without interacting,’ says Chris Mossey, project director of the Deep Underground Neutrino Experiment (DUNE) at Fermilab in Batavia, Illinois.
Neutrinos may be indifferent to us, but we are hardly indifferent to them.
For nearly seven decades, physicists have crafted increasingly elaborate machines to outwit nature and to detect these seemingly undetectable particles. DUNE is the latest and greatest of these devices: a $3.2 billion, 1,300-kilometre-long set of experiments designed to bring neutrinos into view like never before. Mossey, a retired US Navy rear admiral, is part of a global team of more than a thousand researchers working full-tilt to get DUNE up and running by 2031.
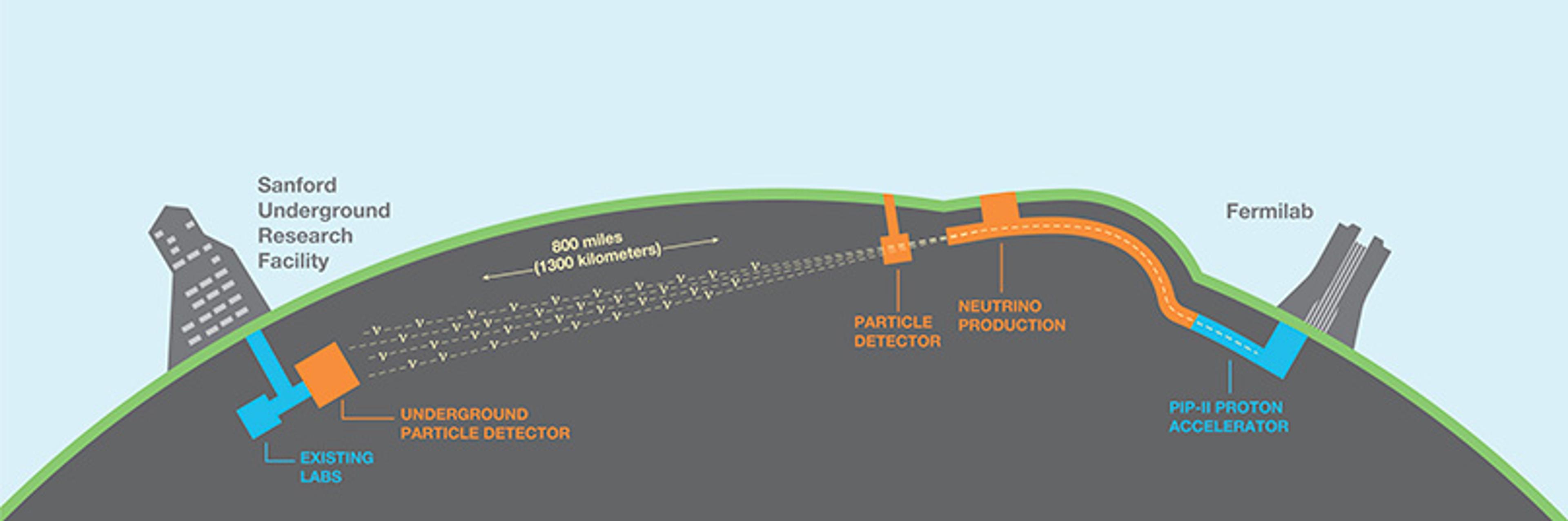
The path of neutrinos in the Deep Underground Neutrino Experiment. A proton beam is produced in Fermilab’s accelerator complex, hits a target and produces a neutrino beam that travels through a particle detector, travelling 800 miles to the detectors at Sanford Underground Research Facility. Courtesy Fermilab
On the face of it, that operation seems like a crazy level of effort to expend on chasing ghosts. The obsession with neutrinos makes sense when you understand what they represent, however. ‘I find myself often explaining to my colleagues: “What’s a neutrino?”’ says Mossey, whose background is not in physics but in supervising huge construction projects for the US Navy. He happily repeats his own neutrino education. ‘The science at DUNE really is amazing. It goes after some of the most fundamental questions that we seek to understand about the Universe: why does matter exist? These are Nobel prize-winning questions if they can be answered.’
According to our current understanding of physics, the Universe was born in an unimaginably hot, intense bath of energy: the Big Bang. That energy should have given rise to precisely equal amounts of matter and antimatter, which should have perfectly annihilated each other. The net result, then, should have been a whole lot of nothingness. Instead, something remarkable happened. Particles of matter outnumbered particles of antimatter, by about one part in 10 billion. That minuscule imbalance made all the difference. The extra particles of matter were enough to create all the galaxies, stars, planets and people we see around us.
Much as the neutrino almost isn’t here, we almost weren’t here, either. We are the cosmic leftovers, the happy result of an unknown kink in the laws of physics.
The peculiar attributes of the neutrino, most notably a protean ability to shapeshift into different versions of itself, could expose the crucial asymmetry that allowed matter to dominate over antimatter. Simply discovering why and how that imbalance occurred would be a breakthrough, but neutrinos may be more than passive messengers about the earliest moments in the life of the Universe. According to some theories, neutrinos actively participated in the genesis of matter, and hence all that followed from the Big Bang. So there is a lot riding on these little particles, and on the giant machines being built to study them.
‘We’d like to know why we’re here – why we exist,’ says Sam Zeller, deputy project director for DUNE at Fermilab. ‘There’s a lot of hope that the results at DUNE will give a definitive answer.’
Physicists have a long history of pinning their grand hopes on the neutrino. In fact, they’ve been doing it since long before anyone knew that these particles even existed.
Starting in 1914, the English physicist James Chadwick began noticing that certain types of radioactive atoms, such as carbon-14, seemed to be misbehaving. During a type of spontaneous nuclear reaction called beta decay, the nuclei of these atoms shoot out high-speed electrons. In theory, the emitted electrons should always emerge the exact same way, carrying away precisely as much energy as is lost from the nucleus. In reality, the electrons always fell short. Even worse, they fell short in an inconsistent way – sometimes by a little, sometimes by a lot.
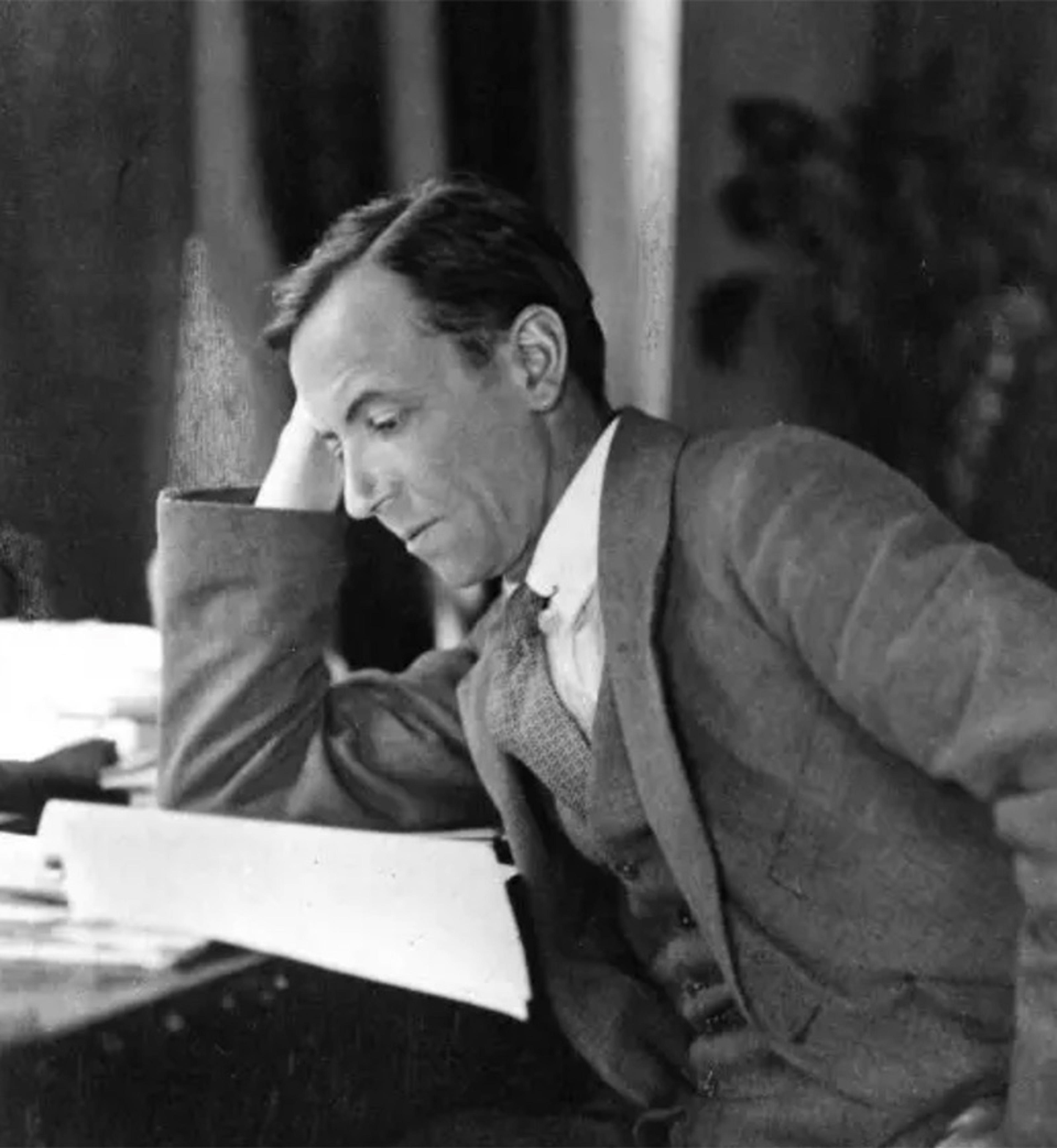
James Chadwick in the early 1930s. Courtesy the Linda Hall Library
Chadwick’s discrepancy carried a disturbing implication: the radioactive atoms seemed to be violating conservation of energy – perhaps the most fundamental law in all of physics, stating that energy cannot be created or destroyed. It was such a shocking result that the renowned Danish physicist Niels Bohr suggested the familiar laws of physics might no longer hold at subatomic scales. Maybe conservation of energy wasn’t a hard-and-fast rule in quantum physics, but more of a statistical average. In this view, a little energy can be destroyed over here or created over there, as long as the overall ledger balances out.
Many of Bohr’s colleagues simply held out hope that Chadwick had made a measurement error. By the time Chadwick published an exhaustive investigation of beta decay in 1922, though, the discrepancy became undeniable and the search for an explanation more urgent.
They rejected the paper, as ‘it contained speculations too remote from reality to be of interest to the reader’
Finally, in December 1930, the Austrian physicist and quantum pioneer Wolfgang Pauli offered what he called a ‘desperate remedy’ to rescue the law of conservation of energy. His idea was so bizarre that he presented it as a whimsical letter written to a group of nuclear physicists meeting in Tübingen, Germany, addressed to ‘Liebe Radioaktive Damen und Herren’ (‘Dear radioactive ladies and gentlemen’). What if, Pauli suggested, an invisible, uncharged particle appears out of nowhere at the moment of beta decay? And what if that wondrous, hypothetical particle then carries away exactly the amount of energy needed to make everything balance out?
At the time, physicists knew of only two subatomic particles, the proton and the electron, and conjuring up a completely new, undetectable particle to explain away a peculiar observation seemed just as outrageous as discarding the principle of conservation of energy. One of the few people to take Pauli’s idea seriously right off the bat was Enrico Fermi, the Italian-born physicist who later gained fame as the creator of the first nuclear reactor. Fermi developed a more comprehensive theoretical description of Pauli’s particle, and in 1934 sent a paper describing his ideas to the journal Nature. The editors rejected the paper, reportedly because ‘it contained speculations too remote from reality to be of interest to the reader’.
But the neutrino proved stubbornly persistent. Continued experiments showed that the electrons emitted during beta decay followed a specific energy pattern, consistent with the presence of an unseen particle rather than with an unconstrained violation of conservation of energy. In 1938, The New York Times declared that the neutrino is ‘no mere hypothesis’, capturing the new consensus view in physics. Even then, Pauli realised that his particle would be extremely difficult to detect – so difficult, that he bet a case of Champagne to anyone who could do the job.
Pauli’s Champagne remained on ice for nearly two decades until the physicists Frederick Reines and Clyde Cowan, Jr, of Los Alamos National Laboratory, became determined to track down the neutrino once and for all (‘Because everybody said you couldn’t do it,’ in Reines’s words). In the early 1950s, Reines and Cowan realised that the best places to look for neutrinos would be where there are a tremendous number of neutrino-spewing nuclear reactions: close to an atomic bomb or a nuclear reactor. They briefly considered placing neutrino detectors next to a bomb test at Los Alamos before switching to a steadier and less lethal source: a US military nuclear reactor at the Savannah River Plant in South Carolina.
For their search, which they nicknamed ‘Project Poltergeist’, Reines and Cowan set up a 1,400-litre detector filled with water and cadmium chloride. On the highly, very, extremely rare occasions when a neutrino would collide with a cadmium atom, it would emit a detectable gamma ray. Using this setup, the determined duo finally found the definitive signal of neutrinos fleeing the Savannah River reactor. On 14 June 1956, they sent a telegram to Pauli: ‘We are happy to inform you that we have definitely detected neutrinos from fission fragments.’ Pauli wrote back: ‘Everything comes to him who knows how to wait.’
And so the neutrino went from a theory to a tangible object.
Now that neutrinos were detectable (albeit barely) in a controlled experiment, another obsessive physicist wanted to study them in the wild. He sensibly set his sights on the largest neutrino-spurting nuclear reactor in the neighbourhood, the Sun. Starting in 1965, the physicist Raymond Davis, Jr persuaded the powers at Brookhaven National Lab to fund the world’s first neutrino telescope. It was a ‘telescope’ only in the loosest sense of the term, since conventional mirrors and lenses are irrelevant to neutrinos. For his detector, Davis used 100,000 gallons of chlorine-rich drycleaning fluid, which he pumped into a cavern of the former Homestake Gold Mine in Lead, South Dakota – the same site, not coincidentally, where the DUNE experiment is currently taking shape.
The vast majority of solar neutrinos flew right through the drycleaning fluid, the same way that they fly through everything else. As with the Reines and Cowan experiment, Davis was relying on sheer numbers to help him. All he needed was a few passing neutrinos to crash into a few of the chlorine atoms among the billion billion billion of them in his tank. When that happened, the chlorine atoms would transform into argon atoms, which Davis could then detect and count. His painstaking effort worked, but only sort of: he got his expected argon atoms, but the resulting numbers seemed all wrong. Davis kept refining and calibrating his experiment until, in 1975, it became clear that the observed flux of neutrinos from the Sun was just one-third of the expected value. The other two-thirds of the particles were AWOL.
Either physicists had misunderstood how the Sun shines, Davis concluded, or they had underestimated how tricky neutrinos are.
Arthur McDonald, an astrophysicist at Queen’s University in Canada, decided to bet big on neutrinos being tricky, and helped design an experiment to prove it. As with all things neutrino, McDonald’s proposed project was a massive undertaking. In the 1980s, he and his colleagues approached the Canadian government with an outrageous request: ‘Do you think we could borrow 4,000 tons of heavy water to do a measurement of neutrinos from the Sun?’ he recalls. ‘At that point, it was about 1.2 billion bucks worth.’
What if neutrinos could change their identities on the fly, oscillating from one form to another?
McDonald was inspired by recent discoveries indicating that the neutrino is not just a single type of particle, but rather a whole family. By the mid-1970s, physicists had deduced that neutrinos come in at least three distinct varieties, or flavours: electron-neutrinos, muon-neutrinos, and tau-neutrinos. Nobody could explain exactly how one kind of ghost particle could have three different ghostly identities, but theory implied that each type of neutrino paired uniquely with one corresponding fundamental particle: the electron, muon, and tau particles.
That number three caught physicists’ attention, suggesting an explanation for the puzzling shortage of neutrino detections in Davis’s experiment. Perhaps the other two-thirds of the expected neutrinos weren’t truly missing, but merely hiding. Physics models indicated that the Sun should produce one flavour of neutrino only – the electron variety – and that’s the only flavour that Davis’s experiment could detect.
But what if, some theorists suggested, neutrinos could change their identities on the fly, oscillating from one form to another? This idea, called the MSW (Mikheyev-Smirnov-Wolfenstein) effect, did not jibe with the behaviour of any other type of particle, but it did have an appealing logic. With three options to choose from, just a third of the solar neutrinos would retain their electron-neutrino identities by the time they reached Earth. And only that third would register in Davis’s detector, exactly matching what he found.
McDonald had to prove that neutrinos really did oscillate in this duplicitous (or rather, tri-plicitous) way. To his amazement and delight, the Canadian government came back with an offer of 1,000 tons of heavy water, enough to get his experiment started and to establish what became the Sudbury Neutrino Observatory in Ontario. Unlike the chlorine atoms in the Davis experiment, heavy water can interact with all three flavours of neutrinos, which allowed McDonald to keep track of the particles, no matter which guise they adopted. ‘In 2001, 2002, we were able to show that neutrinos from the Sun changed from one of the flavours to the other,’ he says. Thirteen years later, McDonald shared the 2015 Nobel prize for his work.
The discovery of neutrino oscillations was another seemingly arcane physics observation that turned out to have profound implications. Oscillations are possible only if some neutrinos have mass, which came as a shock to theorists: ‘The Standard Model [the prevailing theory of fundamental forces and particles, formalised during the 1970s] didn’t predict neutrinos having mass in any way,’ says Alexandre Sousa of the University of Cincinnati, who wields the cool title of Beyond the Standard Model Physics Co-Convener for the DUNE experiment. Where that mass comes from, and what it says about the nature of mass in general, remain active and contentious topics of research.
More important, neutrino oscillations revealed that neutrinos could be used as sensitive probes of natural symmetries – laws of behaviour that remain constant even when you switch one basic condition. For instance, the laws of physics appear the same when you look in a mirror (light is still light, objects still fall down, and so on). The same mirror-image consistency generally holds true for individual particles, a property called ‘parity symmetry’. Particles of matter and particles of antimatter should also behave alike except for the reversal of their electric charges, a property called ‘charge symmetry’. For much of the past century, physicists have heavily relied on symmetries like these to make sense of the rules governing reality.
Neutrinos don’t obey those symmetry rules, however. In particular, they violate the combination of charge and parity symmetry – a combo called, naturally, ‘CP symmetry’. The manner in which neutrinos shift from one flavour to another depends sensitively on exactly how, and how much, they violate CP symmetry. And CP symmetry just so happens to influence the balance between matter and antimatter. The only way to get a universe full of matter (and the good things that come with it, from galaxies to people) is by violating this specific symmetry. Find the breakdown in CP symmetry within the Universe, then, and you might be able to figure out where all this stuff came from.
With neutrinos, ‘you can measure a value for this CP violation,’ Sousa says. ‘If that value is large, then in theory it could explain everything.’ That is where DUNE comes in.
Befitting its grand size and budget, DUNE is a multilayered physics experiment, designed to scrutinise every aspect of neutrino behaviour with unprecedented precision. But above all, it is intended to investigate that vexing question of cosmic origins: why is there something rather than nothing? Our best hope for getting a meaningful answer is to study neutrino oscillations at a level of precision never before possible. To that end, DUNE will incorporate techniques and technologies from all the neutrino experiments that have come before, and vastly expand on them.
The engineering required to make DUNE work is comically out of proportion to the infinitesimal particles it will study. Mossey chuckles in disbelief at the scale of the project: neutrino detectors that measure five stories tall and 200 feet long, sheltered a mile underground, containing nearly 70,000 tons of cryogenic liquid argon. In February 2024, Mossey’s team completed the excavation of the primary workspace for DUNE, three enormous caverns to house those enormous detectors. That task alone required excavating 800,000 tons of rock. ‘And all this had to be done through a mineshaft that’s five feet wide,’ he says. ‘Think of the hoist, think of the power, think of the ventilation.’
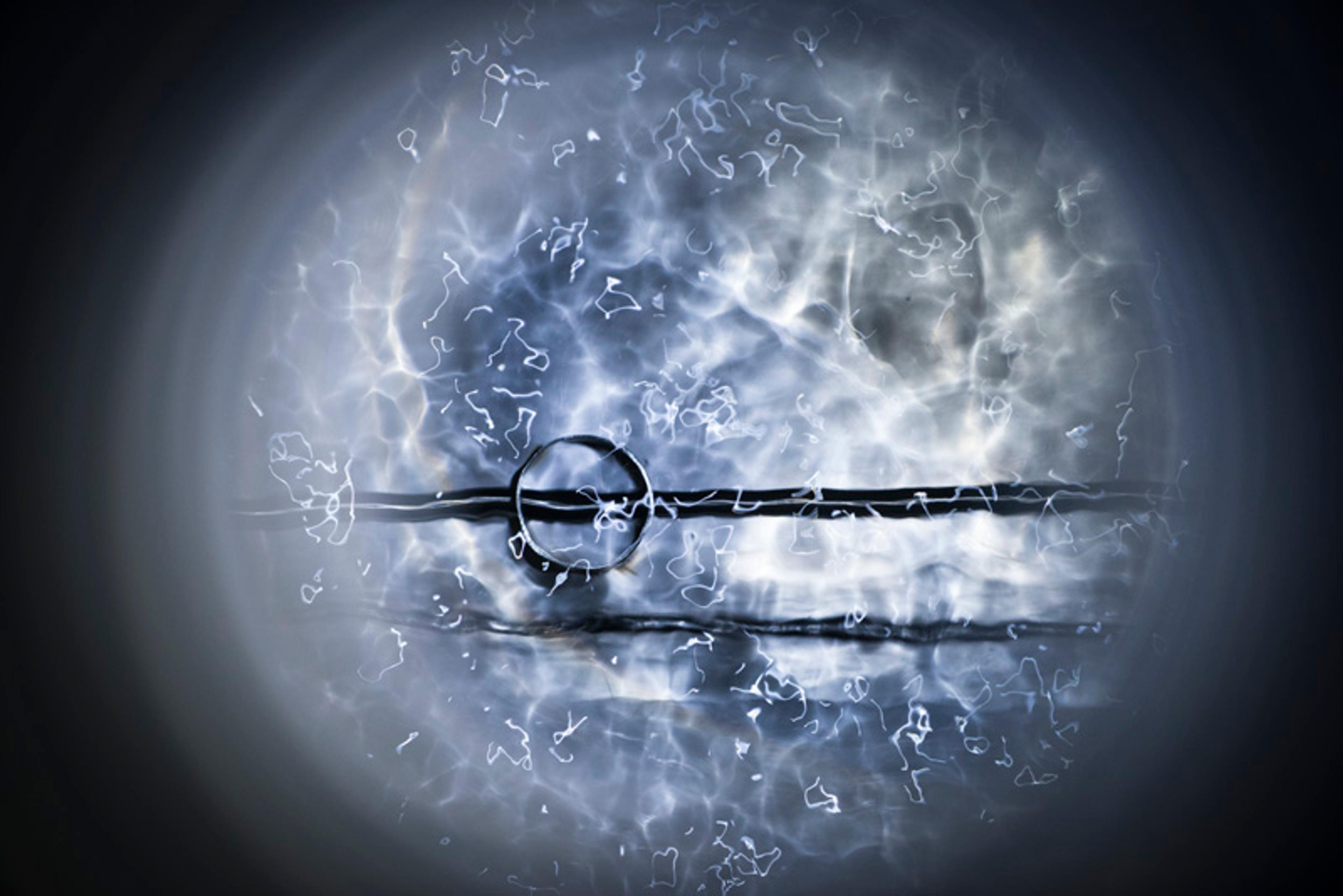
Shimmering liquid argon inside a 35-ton-capacity prototype cryostat used for early tests of LBNF/DUNE. Courtesy Fermilab/Reidar Hahn
When DUNE fully activates in 2031, it will generate ‘the most intense neutrino beam in history’, Zeller says, a 1.2-megawatt neutrino blast designed to expose those ghost particles once and for all. Creating that beam will entail a whole other, marvellously elaborate set of engineering tricks designed to manipulate particles rather than rocks – a quantum Rube Goldberg machine.
Each round of science at DUNE begins at Fermilab – the long-running particle physics facility outside Chicago – where a new particle accelerator, called the Proton Improvement Plan II (PIP-II), shoots out a beam of high-energy protons. Forty-one huge magnets, mostly made at the Bhabha Atomic Research Centre in India, focus and align the protons so that they hit a carefully crafted, 1.5-metre-long cylindrical graphite target. The bombarded target unleashes a spray of particles called pions and kaons. Those particles, in turn, are guided by magnets through a 200-metre-long, helium-filled tunnel, where they decay to produce muon neutrinos. That last step, at last, creates an intense neutrino searchlight that is aimed toward the main detectors in South Dakota.
As far as neutrinos are concerned, our planet is transparent
The DUNE searchlight will fire off neutrinos in pulses lasting just a millisecond or so, once every few seconds, over and over, for a decade or more. ‘They come in short bursts so that you can clearly identify them,’ Zeller says. Otherwise, DUNE’s sensitive detectors could confuse human-made neutrinos with the random ones that are flying around all the time from the rest of the Universe.
At the very beginning of their journey, just 574 metres from the source at Fermilab, the neutrinos get their first physical exam as they pass through a small experiment called the Near detector. Small is a relative term, in this case: the Near detector consists of a movable, 300-ton liquid argon neutrino collector located some 200 feet underground. A core part of its job is to measure the properties of the outgoing beam in support of the main DUNE experiment, but the Near detector will also perform scientific measurements of its own, mostly searching for a possible fourth type of neutrino called a sterile neutrino.
After fleeing the Near detector, the neutrinos keep going at nearly the speed of light, unwavering in their path, straight through Earth. As far as neutrinos are concerned, our planet is transparent. (‘We had a reporter from a tunnelling magazine who was very disappointed that we don’t need to make an 800-mile-long tunnel from Fermilab,’ Mossey says.) About 1/250th of a second later, the particles reach their primary destination: DUNE’s humongous Far detector at the Sanford Underground Research Facility (SURF), located 1.5 kilometres beneath South Dakota, right next to the site of Davis’s old solar-neutrino experiment.
When a passing neutrino beats the astronomical odds and collides with an argon atom in DUNE’s detectors, it sets off a chain reaction. As with all things neutrino, the process is hardly straightforward. The neutrino stimulates the emission of one (or more) charged particle, typically a muon; those particles, in turn, knock loose a series of electrons from neighbouring argon atoms. Then a network of digital sensors detects the electrons, noting their precise energies and locations, to reconstruct the details of the original neutrino culprit in glorious 3D. In the end, humans will be able to ‘see’ neutrinos like never before.
For neutrino researchers who are used to waiting days or months for a single detection, DUNE will be a revelation, delivering perhaps 50 hits a millisecond. ‘It’s something of the likes we’ve not had available to us. You see all the different particle tracks originating from a neutrino colliding with an argon nucleus,’ Zeller says. Researchers will log each event and tag it: what type of neutrino was it; what was its energy; what were its properties?
DUNE will also create a physics community like none before. Davis operated nearly as a one-man band. The DUNE experiment will be more like a neutrino Woodstock. Mary Bishai of Brookhaven National Lab, who started working on DUNE before it even had its name and who now serves as an official spokesperson for the project, has been amazed – and slightly overwhelmed – to watch a sprawling collaboration grow around it. ‘You have 1,400 people, and everybody answers to a different university or lab. It’s a lot of interacting physicists, like herding cats,’ she says wryly. ‘What keeps us working together is the love of the science.’
After all the tunnelling, particle smashing and beam shooting is accomplished, the actual data output at the end of DUNE will essentially be an interference pattern of neutrino interactions – like waves rippling into each other on a lake, their crests adding to or cancelling each other – but constructed from swarms of invisible particles. The three flavours of neutrino will oscillate and interact with each other, creating analogous patterns of neutrino type and intensity. By adjusting DUNE’s beam and detectors, researchers will gradually map those patterns and build up the most complete picture ever of how neutrinos behave.
‘You create muon neutrinos at Fermilab. Then 5 per cent of them are going to oscillate into electron neutrinos, and the rest oscillate into tau neutrinos’ by the time they reach the Far detector, Sousa says. The resulting oscillation pattern, called ‘the mixing angle’, is between the three different neutrino identities. ‘DUNE will measure that mixing angle, and all the physics linked to it,’ Sousa explains. The mixing angle is a set of numbers that indicate how far nature deviates from a simple, mathematically idealised form of symmetry – the kind of sad symmetry that would have led to an empty, barren reality.
While DUNE is busy looking for oscillating neutrinos, it will be taking on many other physics jobs as well. It will search for hypothetical, heavy neutrinos that could explain the unseen dark matter that appears to outweigh all the visible matter in the Universe. These ‘sterile’ neutrinos, if they exist, must be even more inert than the regular kind; they could hide an entire shadow realm of previously unseen physics. The DUNE detectors will function as an ultra-sensitive neutrino telescope, a greatly enhanced version of the experiment Davis began at the same site in the 1960s. If a supernova blows up anywhere in our galaxy, scientists will know it, and will get a unique look into the heart of a dying star – a view that only neutrinos can provide. ‘We would learn so much,’ Sousa says.
DUNE seems like a modern cathedral – a place of congregation where people seek enlightenment
By the end of the DUNE run, sometime around 2040, we should learn how the three known types of neutrinos behave, how they interact, and what masses they have. We may learn the identity of the dark matter that binds together distant galaxies. Above all, we should have a true measure of mixing angles and CP violation, which will tell us if we’ve found the one-part-in-10-billion imbalance that explains why we are here.
Particle physicists will be looking very closely at the exact values of the measured mixing angle for even deeper clues about our origin: not just why matter exists, but where it all came from. If neutrinos display just the right level of imbalance, that finding would support a wild theory called the ‘seesaw mechanism’, which posits that the neutrinos we see today are the meek descendants of ultramassive neutrinos that existed right after the Big Bang. Those bruisers would have been a quadrillion times as massive as a proton; as they decayed, they could have become the direct source of all the atoms that we see today. If so, we are all the progeny of neutrinos.
There would also be the philosophical and sociological satisfaction of drawing together theorists, technicians and hands-on particle physicists from more than 35 countries, working together for more than two decades, to solve an impractical but profoundly personal question: why is there something rather than nothing? ‘How much better a job could you possibly have than to answer a question that absolutely no one on the planet knows the answer to?’ Zeller asks. In that light, DUNE seems less like a piece of machinery than a modern cathedral – a place of congregation where people seek enlightenment, inspired by a shared goal that transcends individuals and that spans generations.
Mossey places the mystery of the neutrino on an even longer evolutionary timescale. ‘You’ve got 65 billion of them going through every square centimetre of your body every second, all your life, going back a 100,000 years to when the first humans walked the Earth,’ he says. ‘Something that ubiquitous has got to have an important role in the Universe, and somehow we’ve got to figure out what it is.’
This Essay was made possible through the support of a grant to Aeon Media from the John Templeton Foundation. The opinions expressed in this publication are those of the author and do not necessarily reflect the views of the Foundation. Funders to Aeon Media are not involved in editorial decision-making.