Humans are shaping the evolutionary future of life on Earth. We’re not only causing mass extinctions, we’re also forcing animals, plants and fungi to adapt to our manufactured world: city birds, for instance, are now singing higher notes, since the pitch seems to help their song carry over the sound of traffic. But while cultural knowledge and engineering have upgraded the human capacity to catalyse environmental change, the proclivity is common to all species. Scatter a few bacteria in a Petri dish and they will produce nutrient-rich byproducts that new bacteria strains can exploit, rapidly causing a multitude of diverse microbial populations to evolve. Beavers create ponds that are used as breeding grounds for ducks; web spiders make retreats that are exploited as hibernation sites by earthbound insects; plants modify soils through substances secreted from their roots. The way these organisms change their environment in turn changes the evolutionary pressures that they and others face as they struggle to survive and reproduce. Their actions, in other words, bias what is selected for. This process is known as ‘niche construction’ and all species do it, even if their effects are sometimes more modest and localised than ours.
Yet the theory of niche construction is controversial among evolutionary biologists, partly because natural selection is traditionally believed to work ‘blindly’: it is thought to sculpt organisms over millennia to become adapted to their ecological niches, with no steer from the goals or purposes of organisms. Humans undergo the same sculpting, but rather than evolving to fit a pre-existing niche, it’s widely accepted that we’re active agents who shape the environments to which we adapt. Our brains have evolved to process linguistically encoded information and learned knowledge because we built a rich cultural realm and then adapted to it. We domesticated plants and animals and, by incorporating them into our diets, triggered selection for genes that metabolise these foods. We invented agriculture, which fuelled population growth, inadvertently selecting for genetic resistance to diseases of the crowd such as typhoid or cholera. Our parents don’t just transmit their genes to us; we also inherit the changed world they leave in their wake. This ecological inheritance means that humans don’t evolve in response to a static adaptive landscape, but instead mould that landscape to alleviate or intensify the particular selective pressures it places upon us. ‘[T]he organism influences its own evolution, by being both the object of natural selection and the creator of the conditions of that selection,’ as the evolutionary biologists Richard Levins and Richard Lewontin put it.
Controversy comes from the fact that experts disagree about the extent to which other creatures can also direct evolution by terraforming their adaptive landscapes. The landscape metaphor can be a helpful one for understanding evolutionary processes. You can think of an adaptive landscape as a graph that reveals the relationship between an organism’s fitness – its ability to survive and reproduce – and one or more of the organism’s traits. For instance, in a population of birds, one axis might represent body size, and another tail length (or instead of traits, we can plot the frequency of the associated genes). The landscape is represented by a 3D surface, with the well-adapted birds with good size-and-tail-length combinations living it up on the peaks, while the poorly adapted birds scrape out a living in the valleys. The region with the highest peak represents the creature with the optimal body size and tail, best able to survive. A population of birds will have a range of characteristics, with each combination a different point on the landscape – and through natural selection, the population will gradually converge on the traits best suited to the local habitat, represented on the adaptive landscape as the population climbing a local fitness peak. But there’s more than one way to get to the top of an adaptive peak: you can change your traits to help you reach the summit, or you can move the mountain so that it comes to you.
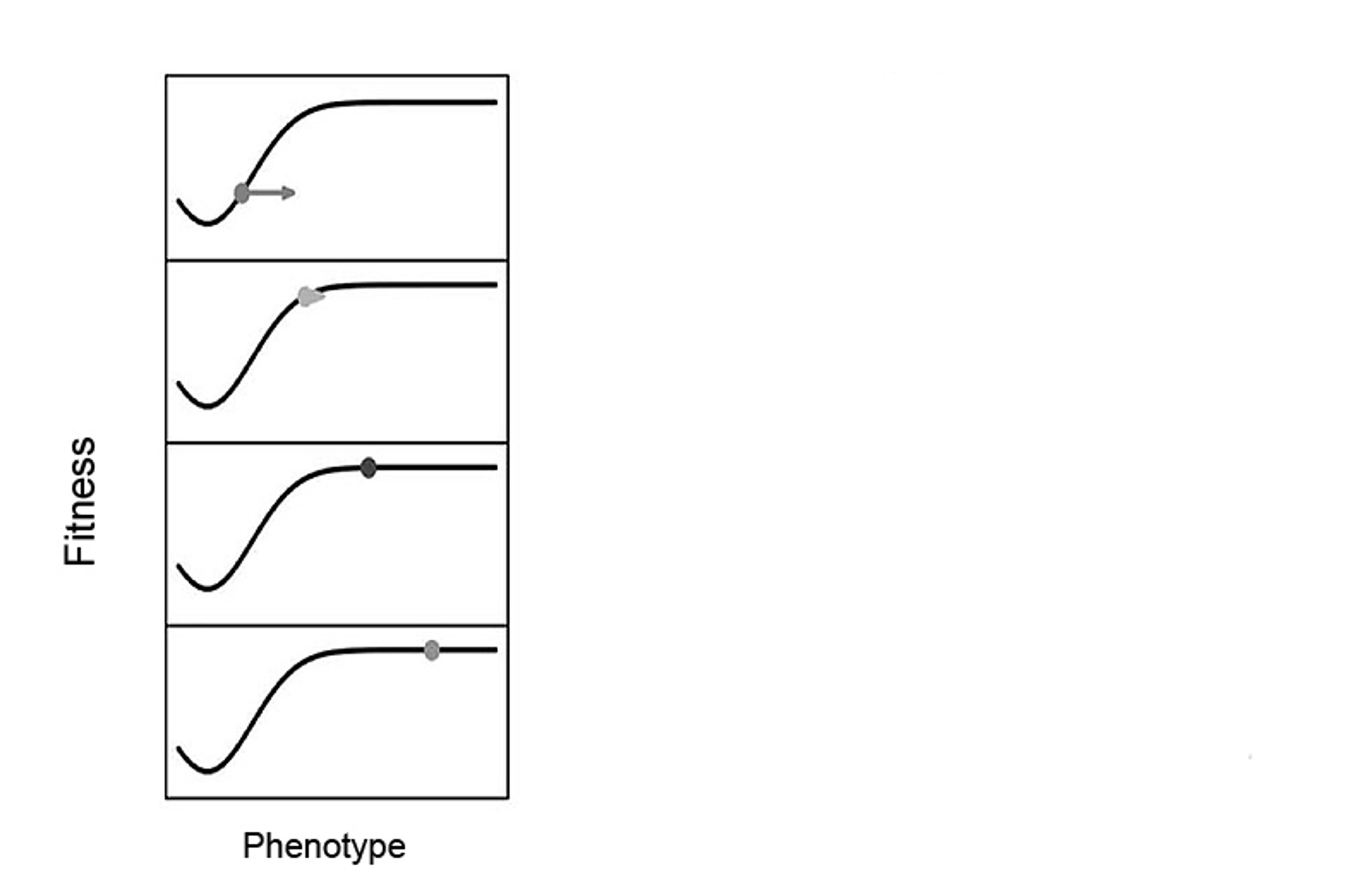
An adaptive landscape that is static, uninfluenced by organisms. In the panel, time moves downwards. Figure adapted from Tanaka et al (2020), Philosophy of Science, 87: 478-98
Small and mighty niche constructors can be found right beneath our feet. The humble earthworm is an ‘ecosystem engineer’ – a species that alters the physical and chemical environment, often in ways that affect ecosystem functioning. Oddly, despite their ecological success, earthworms are actually anatomically ill-suited for life on land. They retain the basic physiology of the freshwater worms from which they evolved, despite living on land for tens – perhaps hundreds – of millions of years. Unlike most terrestrial animals, but in line with freshwater creatures, earthworms produce large quantities of dilute urine, which leaves them highly vulnerable to drying up: if you dig up an earthworm in your garden on a sunny day, it often seems to shrivel up instantaneously. So how could an animal survive for so long on land with such poor structural adaptation?
The answer is niche construction. Rather than adapting their physiology to a terrestrial environment, earthworms manufacture an aquatic world on land. Their success derives directly from their landscape gardening. Earthworms tunnel and burrow in ways that reduce what’s known as the ‘matric potential’ of soil – the ability of the soil to hold on to water. This makes it easier for earthworms to draw water to their bodies, replenishing the water losses caused by their excretions. Earthworms effectively build their own paddling pools to keep themselves moist.
In this way, earthworms deploy a variety of techniques to skew the adaptive landscape in their favour, with effects eerily evocative of the aftermath of a human invasion. European earthworms are now recognised to be the major driving force behind changes in North American forest plant communities – and contrary to the popular image that they’re always good for the soil, earthworms are having a devastating impact on hardwood forests. The original earthworm fauna of North America became virtually extinct during the Pleistocene glaciation, with many ecosystems earthworm-free until European settlers introduced them in the 16th and 17th centuries. European earthworms have subsequently spread dramatically, and their invasion of deciduous forests is accompanied by striking changes in soil properties that leave native plant and animal species reeling.
The worms drag organic matter into the soil, as food or as materials to line their burrows, leading to rapid loss of the litter layer, and resulting in warmer, drier soil surfaces. Their activities alter the rates at which chemical elements such as carbon and nitrogen cycle through the ecosystem, for instance, speeding up the decomposition of plant materials, while their tunnelling and burrowing increases soil aeration and drainage. These changes typically occur within 10 years of invasion, introducing an alternative state that persists for many decades. Forests that historically lacked earthworms contain large amounts of carbon trapped in surface litter. Like human use of fossil fuels, when earthworms eliminate these layers, they release vast amounts of stored carbon into the atmosphere as carbon dioxide, contributing to global warming.
Earthworm invasions trigger ecological cascades that reverberate through forest ecosystems
These changes in habitat quality have a profound impact on other species. Recent studies found that plant diversity declined as the richness of introduced earthworm ecological groups increased, with native plants hardest hit, while soil invertebrates decreased as earthworm biomass rose. Native plants and animals suffer because they’re adapted to an environment without earthworms. At the same time, some species benefit: much like human disturbances, earthworm engineering promotes the invasion and proliferation of fast-growing bacteria, grasses, sedges and some large animals such as deer. Earthworms’ burrows are used as retreats by invertebrates, and even some vertebrates, such as salamanders, while their dunghills (‘middens’) are hotspots of microbe activity. The worms commonly co-occur with invasive shrubs too, such as buckthorn and honeysuckle. That’s partly because earthworms distort the seed bank – the natural storage of seeds in the soil – by eating seeds, choosing them selectively according to traits such as size. Seeds that survive being eaten gather in earthworm casts (worm poo), benefiting from the nutrients and physical protection of this natural fertiliser. Invariably, it’s the non-native plants that reap the advantage.
No surprise, then, that earthworm invasions trigger ecological cascades that reverberate through forest ecosystems. Some researchers are even concerned that earthworms could trigger an ‘invasion meltdown’, where non-indigenous species facilitate one another’s invasion in an accelerating fashion. What’s perhaps less intuitive is that earthworms themselves benefit from their engineering and the invasions it triggers, allowing them to build new fitness peaks on the adaptive landscape. While earthworms promote the growth of invasive shrubs, those shrubs reciprocally facilitate the growth of earthworm populations. Self-perpetuating feedback loops arise because the shrubs produce a higher-quality leaf litter (that is, with a lower carbon-to-nitrogen ratio), favoured by European earthworms. The earthworms effectively engage in farming – preparing the soil, skewing the seed bank and germinating their preferred crops in their middens. They culture similar mutually favourable relationships with deer, pigs and microorganisms, who also benefit from and promote earthworm activity. Interestingly, the diversity of earthworm species correlates with earthworm biomass, suggesting that earthworm engineering benefits other earthworm species, too.
Earthworms also build houses to stack the evolutionary odds in their favour. There are more than 6,000 species of earthworms, which differ considerably in their ecology and behaviour. However, most are not just wandering nomads, roving the soil in search of food and leaving displaced earth behind. Rather, earthworms can construct semi-permanent burrows, which commonly last substantially longer than a lifetime. Charles Darwin himself noted that earthworm burrows aren’t simple excavations, but rather robust vertical tunnels, sometimes more than a metre deep, which he described as ‘lined with cement’. Earthworm ‘cement’ arises from the compaction of secretions and castings produced by the resident worm, and creates a distinctive burrow lining up to a centimetre in thickness, known as a ‘drilosphere’. Each earthworm burrow – the living quarters – opens up on the soil surface with a midden, a kind of compost heap that serves as both kitchen and toilet, comprising a mound of litter collected as food together with earthworm casts. Earthworms are even known to excavate side-burrow nurseries in which cocoons are laid. From the safety of their homes, many earthworms scavenge in the dark on the surface for food and mates, often leaving their tail ends safely attached to the burrow.
Burrowing is an energetically costly activity – much easier to adopt and renovate a previous incumbent’s home than to build your own from scratch. Earthworms aren’t thought to dig burrows wider than their body width, yet small worms are commonly found in large burrows, suggesting either that juveniles inherited a parental burrow or that dispersing individuals will readily set up home in vacant burrows. Long-term field observations reveal that the same burrows are continuously used by worms for periods far longer than a worm lifetime. Unsurprising, then, that earthworms appear to prefer soils previously inhabited by worms over virgin soils.
Improved soils, with ready-built homes and an improved food supply, aren’t all that earthworms bequeath to successive generations. Each earthworm cocoon inherits a community of microorganisms stocked from their parents’ gut microbiome. The environment of the earthworm isn’t just the soil and the creatures around it, but the bacteria, fungi and single-cell eukaryotes inhabiting its digestive system and the surrounding drilosphere. Once again, the arrangement is mutually beneficial: the earthworm constitutes a rich and mobile environment for soil microorganisms, giving them the opportunity to ferment in an oxygen-deprived chamber and propagate through the soil, while the presence of these symbionts increases earthworm fitness.
Just like humans, earthworms have constructed their own niche. Their environments aren’t fixed as rich or poor, but remain dynamic and changeable according to the activity of their inhabitants. Worms aren’t just altering soils – they’re cultivating crops, releasing greenhouse gasses, building homes and aggregating into cities that sprawl out into the countryside, just as we do.
Nor are earthworms the only species that improve their environment through niche construction: marine algae secrete sticky chemicals that bind the sand and stabilise the environment; chaparral and pine trees promote forest fires, which burn off the competition, through dispersing flammable needles, cones and oils; and ant species eliminate trees unsuitable for their colonies by spraying them with acid herbicides or cultivating fungus crops.
Does the way in which animals improve environments in relation to their needs demand new ways of thinking about evolution’s adaptive landscape? From the most radical niche-construction standpoint, the answer is ‘yes’: organisms don’t just adapt, they co-direct evolution. The impact of beavers, for instance, is much more extensive than the ponds that they create. They are fire-fighters and irrigation managers, who drastically alter upstream ecosystems, making them resilient to climate disturbances such as fire and drought. In this way, beavers change their local environment and so modify how selection acts on them and others. They’re part of looping networks of feedback and causation, in which previously selected organisms drive environmental changes, and organism-modified environments subsequently select for changes in organisms.
Niche construction overlaps with several other concepts in ecology and evolution, notably ‘ecosystem engineering’ and ‘eco-evolutionary dynamics’. What’s distinctive about niche construction theory, however, is the claim that environmental modification by organisms (niche construction), and its legacy over time (ecological inheritance), are evolutionary processes – they cause evolutionary change.
Niche construction modifies natural selection in an orderly, directed and sustained manner
What counts as an evolutionary process isn’t settled, though most textbooks have focused on phenomena that directly change gene frequencies – such as natural selection, mutation or random genetic drift (changes in the genetic composition of a population that occur through chance). Going back to the adaptive-landscape metaphor, these are the forces that push populations of organisms up and around the hills and valleys of the landscape. Niche construction theory, in contrast, advocates a broader conception of evolutionary causation.
‘Traditionalists’ would say that, while niche construction ‘can influence or even cause the evolutionary process of natural selection’, it’s not ‘itself an evolutionary process, any more than a changing environment is,’ in the words of the evolutionary biologist Douglas Futuyma. What this perspective omits, and what differentiates niche construction from environmental change more generally, is that niche construction modifies natural selection in an orderly, directed and sustained manner. It doesn’t just change, but directs adaptive evolution, by imposing a statistical bias on the direction and strength of selection. Organisms engineer the adaptive landscape. For instance, an analysis of earthworm invasions on native plant communities in 15 forests across the northeastern United States revealed strikingly consistent patterns. The same earthworm-associated responses were detected at all sites, despite differences in earthworm species and abundance, native plant composition and identity, and geographical region.
Perhaps instead of an adaptive landscape with solid hills and valleys, it would be better to think of evolution in terms of organisms walking across a trampoline, as Lewontin has suggested. However, the earthworm example shows that, as they move through evolutionary time, organisms don’t just suppress their fitness, but they can also improve their environment, and hence their fitness relative to it. This engineering is sufficiently powerful to help determine evolutionary outcomes. For instance, earthworms didn’t move up the fitness peak of a typical land-animal’s physiology. Instead, they took another evolutionary journey to an unlikely fitness peak, representing an aquatic physiology on land.
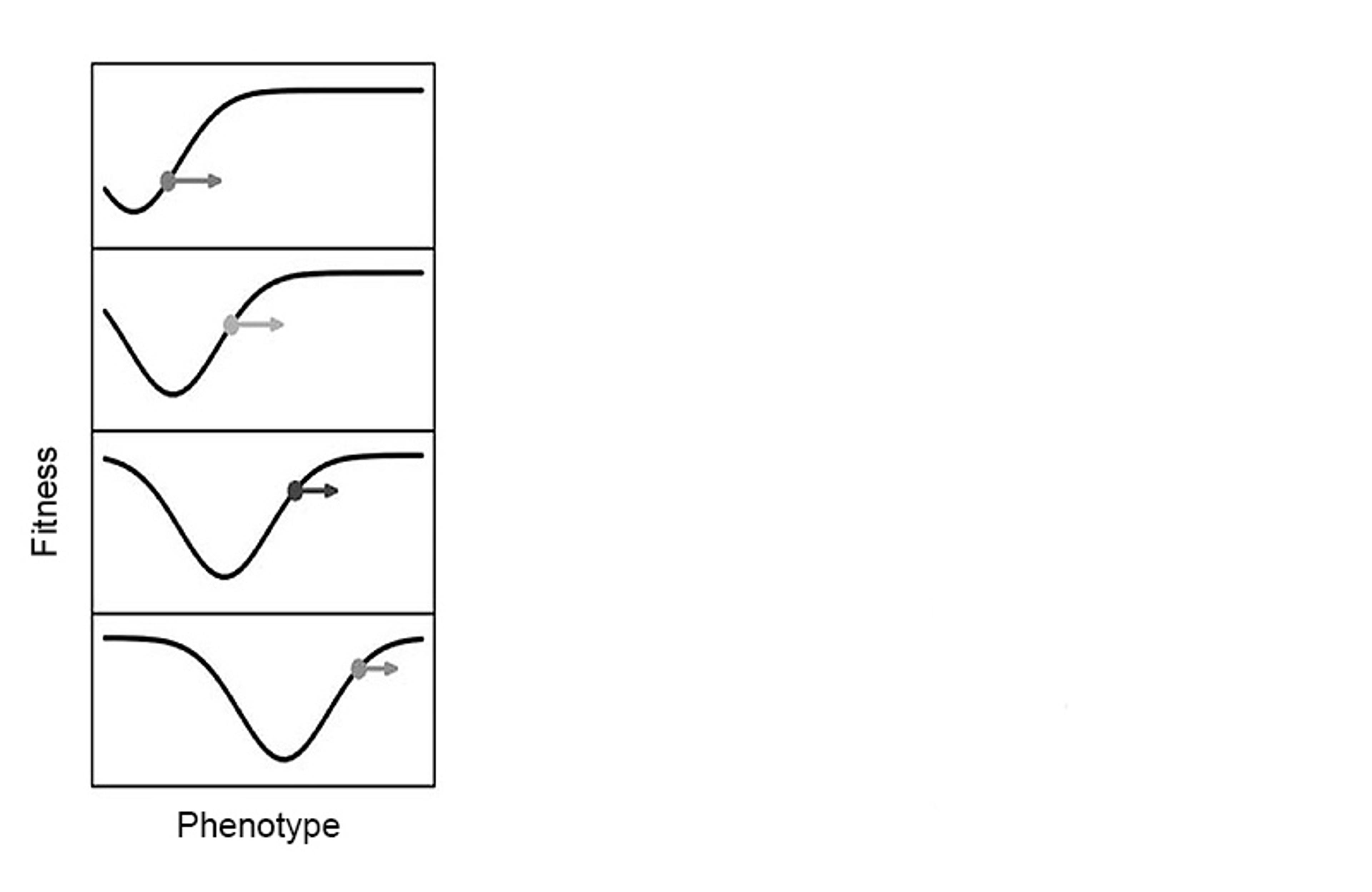
An adaptive landscape depressed by organisms. In the panel, time moves downwards. Figure adapted from Tanaka et al (2020), Philosophy of Science, 87: 478-98
Recently, Mark Tanaka, Peter Godfrey-Smith and Benjamin Kerr – a team of theoretical biologists and a philosopher – devised a novel mathematical framework for modelling natural selection and niche construction. They were able to characterise adaptive evolution on two linked landscapes, rather than a single surface: the evolution of the organism occurs by hill-climbing on an adaptive landscape, and simultaneously the evolution of the environment occurs via hill-climbing on a constructive landscape. It’s the relationship between the two that determines how successful a particular organism is. Just as we can’t adequately understand the evolution of secondary sexual characters, such as peacocks’ tails, without tracking the coevolution of peahens’ mating preferences, so the evolution of traits of which the fitness depends on features of the environment modified by organisms can’t be described without incorporating niche construction. Tanaka and colleagues used their framework of evolution by niche construction to analyse the dynamics of three cases of microbial evolution, in which the microbes engage in different forms of niche construction.
The way natural selection drives populations up adaptive landscapes is described by what’s commonly known as Fisher’s fundamental theorem: the rate of increase in fitness of a population evolving through natural selection is equal to the genetic variance in fitness (that is, the amount of genetic variation that contributes to differences in survival and reproduction). The statistician and geneticist Ronald Fisher assumed that, since populations can’t continue to get fitter indefinitely, the process must be balanced by a corresponding deterioration of the environment. Tanaka and colleagues’ model of coupled landscapes shows mathematically that, under niche construction, this conservation of fitness doesn’t necessarily hold. Organisms can alter their environment in such a way as to improve their absolute fitness (like building mounds on hills), as well as to simultaneously damage their environment and lower their fitness (like digging pits).
The model is relevant to understanding the evolution of earthworms and other species that remodel the environment, including humans. The dramatic success of European earthworms in North American forests is most likely primarily not a manifestation of natural selection adapting earthworms to forest environments, but rather a consequence of the earthworms’ capacity to change forest ecology to better suit themselves. The same point holds for humans. Domesticating plants, tilling the soil, irrigation methods, fertilisers – they all increase the ability of the land to support humans. We have ratcheted up our absolute fitness through repeated bouts of environmental amelioration – as if we built taller and taller towers on the adaptive landscape, and then climbed up them.
The evolutionary ecologists Tobias Uller and Heikki Helanterä devised a way to incorporate niche construction into another general evolutionary model, known as the Price equation, named after its enigmatic author George Price. It’s built on two core processes of evolution: the natural selection of traits over time; and their biased transmission (or inheritance) across generations. Each of these processes can be defined and measured statistically, providing a way to calculate change in traits over time (ie, evolution), and how much either selection or transmission contributes, respectively. The selection term is given by the product of the heritability of the trait (how much genetic differences contribute to variation in a trait) and the selection differential (which measures how much the trait affects fitness). For instance, if big dogs have more puppies than small dogs, but dogs with erect and with pendulous ears have similar numbers of offspring, then size would have a large selection differential and ear shape a small one. The trait of interest can be anything, including fitness. As the heritability is related to the amount of genetic variation, when the focal trait is fitness, the selection term in the Price equation is broadly equivalent to Fisher’s fundamental theorem.
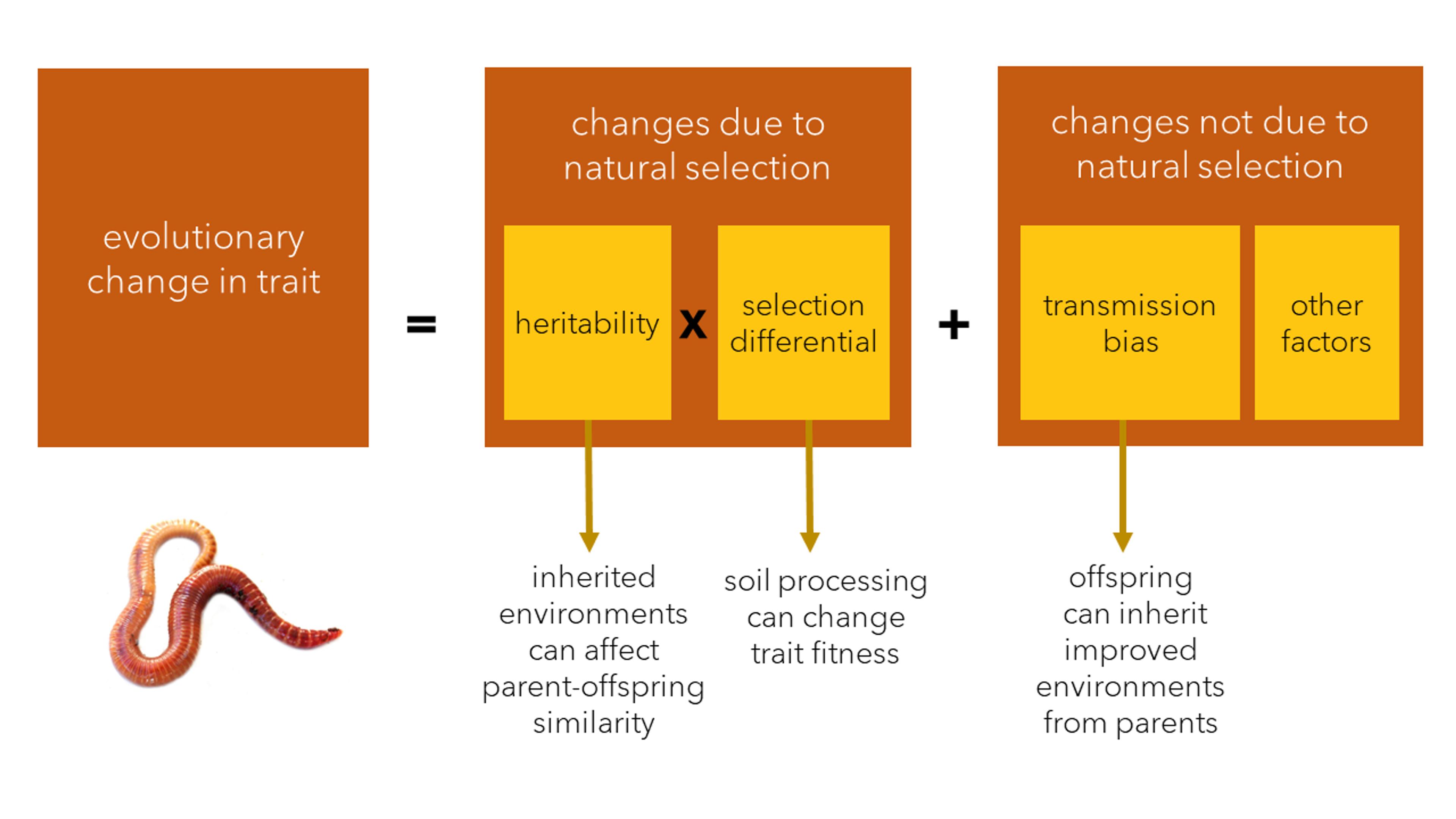
The Price Equation models evolutionary change as a result of natural selection (which can be decomposed into two components: heritability and the selection differential) and non-selection factors such as transmission bias. Niche construction can affect all three components of evolution, with earthworms presenting a prime example. Graphic courtesy the authors
The transmission term, by contrast, represents factors other than direct selection that can affect how the trait would change over time, including biases in what is inherited. Interestingly, Price referred to this as the ‘environmental change term’, which seems particularly apt for the earthworms. Earthworms are not haphazardly changing the soil, sometimes bequeathing a better world to descendants, and sometimes worse; nor are they relentlessly degrading it. Rather, generation after generation, earthworms improve the quality of the soil for themselves, driving up their own fitness through niche construction, and leaving a biased ecological inheritance for their offspring. As with Fisher’s assumption that environments should deteriorate, historically researchers have tended to assume that the transmission-bias term in the Price equation is likely to be negative, when the focal trait is fitness. That’s because mutations are usually bad for organisms, and also because environmental effects are (wrongly) assumed not to be inherited. As a result, the role that processes involved in transmission play in evolution has been comparatively neglected. Where organisms construct or choose better environments for their offspring, they can potentially cause adaptive evolution – even in the absence of natural selection.
Uller and Helanterä use the Price equation to point out further ways in which niche construction can influence evolution. In evolutionary analyses, heritability – how much traits differ because of genetic differences – is often estimated by statistics (eg, parent-offspring regression) that compare the similarity of the trait in parents and offspring (ie, do large dogs have big puppies?) The transmission of organism-modified environments across generations affects how similar offspring are to their parents, often in ways that inflate heritability estimates.
Evolving populations are less like zombie mountaineers, and more like industrious landscape designers
Again, earthworms provide a helpful illustration. Earthworms are slimy because they secrete mucus to protect their outer skin layer, and mucus secretion varies in a manner sensitive to soil properties. Measured across soil conditions that vary in the extent of earthworm processing, there would be strong correlations between the levels of mucus among parents and offspring. However, that’s not because slimy worms pass on ‘excess slime’ genes to their babies. The parent-offspring similarity and appearance of high heritability happens mostly because of ecological not genetic inheritance: offspring ‘inherit’ the soils (and microbiome) of their parents and consequently produce similar amounts of mucus. If researchers investigated how mucus levels respond to natural selection in invading earthworms, they would likely detect a strong shift in the trait – implying selection for low mucus secretion. But in reality, this would be an ecological effect of niche construction as earthworms improve the soil environment for later generations. There has been no genetic response to natural selection, yet systematic and directional change in the trait has occurred, in a manner strongly associated with fitness.
Another way that niche construction can influence evolution is by modifying how the trait affects fitness (ie, changing the selection differential, part of that first selection term in the Price equation). For instance, let the trait be the tendency of earthworms to migrate when they encounter poor soil conditions or low food availability. Experimental investigations also show that earthworms can reduce the need for themselves (and other earthworm species) to change location by improving habitat quality. Rather than moving to richer pastures, they irrigate, fertilise and replant the land, transforming it into prime real estate.
Now consider the fitness of (hypothetical) ‘fast’ and ‘slow’ dispersers in an invading earthworm population. Fast dispersers might colonise the soil, but initially have relatively low fitness as the virgin soil is of poor quality. However, as they improve their environment, they attract more worms, as well as the beneficial (to them) invasive shrubs and animals, and so their fitness improves further. Eventually, the earthworm population becomes so dense that their fitness declines through competition and overexploitation, and a new wave of dispersal is triggered. Slow dispersers, by contrast, initially have higher relative fitness than the colonisers. However, as the soil quality of the invaded forest improves, slow dispersers’ relative fitness declines, until they too disperse. Fast and slow dispersers are locked into a cyclical battle, driven by niche construction and competition. Returning to the fitness-landscape metaphor, here the landscape is like a seesaw: low dispersal is favoured but, as fast dispersers improve the soil, the plank tips the other way and high dispersal is a fitness peak, before competition flips it back again.

An adaptive landscape seesaw, the direction of adaptation changed by organisms. In the panel, time moves downwards. Figure adapted from Tanaka et al (2020), Philosophy of Science, 87: 478-98
All this formal mathematical analysis has been immensely helpful to clarify understanding of the consequences of how organisms modify their environments. To grasp adaptive evolution, researchers need to understand not just how niche construction evolves through natural selection, but how the environmental sources of natural selection are themselves transformed by niche construction. We need to extend the adaptive-landscape metaphor to capture the active, directed environmental change that organisms perform. Evolving populations are less like zombie mountaineers mindlessly climbing adaptive peaks, and more like industrious landscape designers, equipped with digging and building apparatuses, remodelling the topography to their own ends. At a time when human niche construction and ecological inheritance are ravaging the planet’s ecology and driving a human population explosion, understanding how organisms retool ecology for their own purposes has never been more pressing.
To learn more, go to Niche Construction