A couple of years ago, at a massive conference of neuroscientists — 35,000 attendees, scores of sessions going at any given time — I wandered into a talk that I thought would be about consciousness but proved (wrong room) to be about grasshoppers and locusts. At the front of the room, a bug-obsessed neuroscientist named Steve Rogers was describing these two creatures — one elegant, modest, and well-mannered, the other a soccer hooligan.
The grasshopper, he noted, sports long legs and wings, walks low and slow, and dines discreetly in solitude. The locust scurries hurriedly and hoggishly on short, crooked legs and joins hungrily with others to form swarms that darken the sky and descend to chew the farmer’s fields bare.
Related, yes, just as grasshoppers and crickets are. But even someone as insect-ignorant as I could see that the hopper and the locust were radically different animals — different species, doubtless, possibly different genera. So I was quite amazed when Rogers told us that grasshopper and locust are in fact the same species, even the same animal, and that, as Jekyll is Hyde, one can morph into the other at alarmingly short notice.
Not all grasshopper species, he explained (there are some 11,000), possess this morphing power; some always remain grasshoppers. But every locust was, and technically still is, a grasshopper — not a different species or subspecies, but a sort of hopper gone mad. If faced with clues that food might be scarce, such as hunger or crowding, certain grasshopper species can transform within days or even hours from their solitudinous hopper states to become part of a maniacally social locust scourge. They can also return quickly to their original form.
In the most infamous species, Schistocerca gregaria, the desert locust of Africa, the Middle East and Asia, these phase changes (as this morphing process is called) occur when crowding spurs a temporary spike in serotonin levels, which causes changes in gene expression so widespread and powerful they alter not just the hopper’s behaviour but its appearance and form. Legs and wings shrink. Subtle camo colouring turns conspicuously garish. The brain grows to manage the animal’s newly complicated social world, which includes the fact that, if a locust moves too slowly amid its million cousins, the cousins directly behind might eat it.
How does this happen? Does something happen to their genes? Yes, but — and here was the point of Rogers’s talk — their genes don’t actually change. That is, they don’t mutate or in any way alter the genetic sequence or DNA. Nothing gets rewritten. Instead, this bug’s DNA — the genetic book with millions of letters that form the instructions for building and operating a grasshopper — gets reread so that the very same book becomes the instructions for operating a locust. Even as one animal becomes the other, as Jekyll becomes Hyde, its genome stays unchanged. Same genome, same individual, but, I think we can all agree, quite a different beast.
Why?
Transforming the hopper is gene expression — a change in how the hopper’s genes are ‘expressed’, or read out. Gene expression is what makes a gene meaningful, and it’s vital for distinguishing one species from another. We humans, for instance, share more than half our genomes with flatworms; about 60 per cent with fruit flies and chickens; 80 per cent with cows; and 99 per cent with chimps. Those genetic distinctions aren’t enough to create all our differences from those animals — what biologists call our particular phenotype, which is essentially the recognisable thing a genotype builds. This means that we are human, rather than wormlike, flylike, chickenlike, feline, bovine, or excessively simian, less because we carry different genes from those other species than because our cells read differently our remarkably similar genomes as we develop from zygote to adult. The writing varies — but hardly as much as the reading.
This raises a question: if merely reading a genome differently can change organisms so wildly, why bother rewriting the genome to evolve? How vital, really, are actual changes in the genetic code? Do we always need DNA changes to adapt to new environments? Are there other ways to get the job done? Is the importance of the gene as the driver of evolution being overplayed?
You’ve probably noticed that these questions are not gracing the cover of Time or haunting Oprah, Letterman, or even TED talks. Yet for more than two decades they have been stirring a heated argument among geneticists and other evolutionary theorists. As evidence of the power of rapid gene expression and other complex genomic dynamics mounts, these questions might (or might not, for pesky reasons we’ll get to) begin to change not only mainstream evolutionary theory but our more everyday understanding of evolution.
Twenty years ago, phase changes such as those that turn grasshopper to locust were relatively unknown, and, outside of botany anyway, rarely viewed as changes in gene expression. Now, notes Mary Jane West-Eberhard, a wasp researcher at the Smithsonian Tropical Research Institute in Panama, sharp phenotype changes due to gene expression are ‘everywhere’. They show up in gene-expression studies of plants, microbes, fish, wasps, bees, birds, and even people. The genome is continually surprising biologists with how fast and fluidly it can change gene expression — and thus phenotype.
These discoveries closely follow the recognition, during the 1980s, that gene-expression changes during very early development — such as in embryos or sprouting plant seeds — help to create differences between species. At around the same time, genome sequencing began to reveal the startling overlaps mentioned above between the genomes of starkly different creatures. (To repeat: you are 80 per cent cow.)
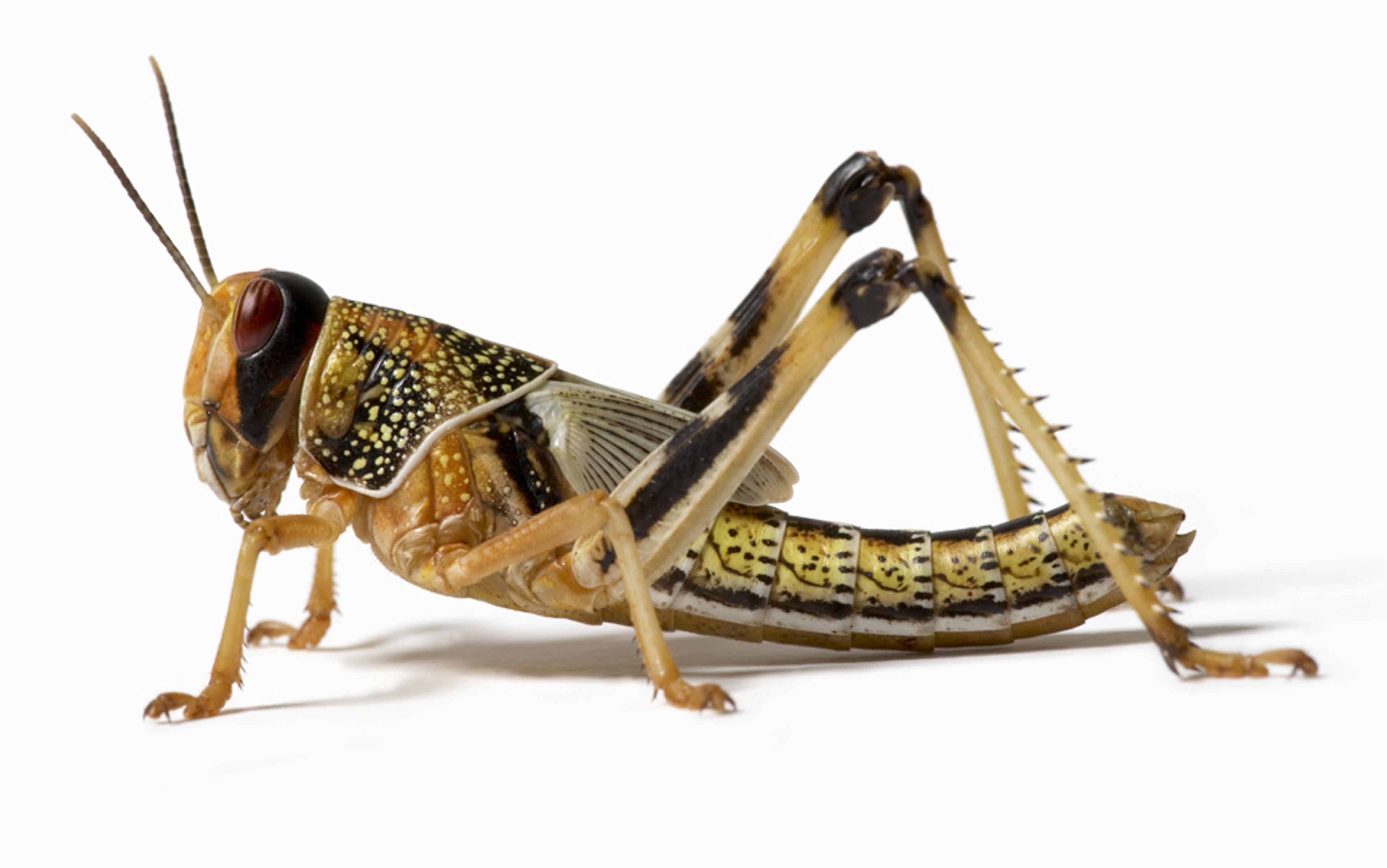
Shapeshifter: the locust. Photo by Ocean/Corbis
Gregory Wray, a biologist at Duke University in North Carolina who studies fruit flies, sees this flexibility of genomic interpretation as a short path to adaptive flexibility. When one game plan written in the book can’t provide enough flexibility, fast changes in gene expression — a change in the book’s reading — can provide another plan that better matches the prevailing environment.
‘Different groups of animals succeed for different reasons,’ says Wray. ‘Primates, including humans, have succeeded because they’re especially flexible. You could even say flexibility is the essence of being a primate.’
According to Wray, West-Eberhard and many others, this recognition of gene expression’s power, along with other dynamics and processes unanticipated by mainstream genetic theory through the middle of last century, requires that we rethink and expand the way we view genes and evolution. For a century, the primary account of evolution has emphasised the gene’s role as architect: a gene (or gene variant) creates a trait that either proves advantageous or not, and is thus selected for, changing a species for the better, or not. Thus, a genetic blueprint creates traits and drives evolution.
This gene-centric view, as it is known, is the one you learnt in high school. It’s the one you hear or read of in almost every popular account of how genes create traits and drive evolution. It comes from Gregor Mendel and the work he did with peas in the 1860s. Since then, and especially over the past 50 years, this notion has assumed the weight, solidity, and rootedness of an immovable object.
But a number of biologists argue that we need to replace this gene-centric view with one that more heavily emphasises the role of more fluid, environmentally dependent factors such as gene expression and intra-genome complexity — that we need to see the gene less as an architect and more as a member of a collaborative remodelling and maintenance crew.
‘We have a more complicated understanding of football than we do genetics and evolution. Nobody thinks just the quarterback wins the game’
They ask for something like the rejection a century ago of the Victorian-era ‘Great Man’ model of history. This revolt among historians recast leaders not as masters of history, as Tolstoy put it, but as servants. Thus the Russian Revolution exploded not because Marx and Lenin were so clever, but because fed-up peasants created an impatience and an agenda that Marx articulated and Lenin ultimately hijacked. Likewise, D-Day succeeded not because Eisenhower was brilliant but because US and British soldiers repeatedly improvised their way out of disastrously fluid situations. Wray, West-Eberhard and company want to depose genes likewise. They want to cast genes not as the instigators of change, but as agents that institutionalise change rising from more dispersed and fluid forces.
This matters like hell to people like West-Eberhard and Wray. Need it concern the rest of us?
It should. We are rapidly entering a genomic age. A couple of years ago, for instance, I became one of what is now almost a half-million 23andMe customers, paying the genetic-profiling company to identify hundreds of genetic variants that I carry. I now know ‘genes of interest’ that reveal my ancestry and help determine my health. Do I know how to make sense of them? Do they even make sense? Sometimes; sometimes not. They tell me, for instance, that I’m slightly more likely than most to develop Alzheimer’s disease, which allows me to manage my health accordingly. But those genes also tell me I should expect to be short and bald, when in fact I’m 6’3” with a good head of hair.
Soon, it will be practical to buy my entire genome. Will it tell me more? Will it make sense? Millions of people will face this puzzle. Along with our doctors, we’ll draw on this information to decide everything from what drugs to take to whether to have kids, including kids a few days past conception — a true make-or-break decision.
Yet we enter this genomic age with a view of genetics that, were we to apply it, say, to basketball, would reduce that complicated team sport to a game of one-on-one. A view like that can be worse than no view. It tempts you to think you understand the game when you don’t. We need something more complex.
‘And it’s not as if people can’t handle things more complex,’ says Wray. ‘Educated people handle ideas more complex than this all the time. We have a more complicated understanding of football than we do genetics and evolution. Nobody thinks just the quarterback wins the game.
‘We’re stuck in an outmoded way of thinking that should have fallen long ago.’
This outmoded thinking grew from seeds planted 150 years ago by Gregor Mendel, the monk who studied peas. Mendel spent seven years breeding peas in a five-acre monastery garden in the town of Brno, now part of the Czech Republic. He crossed plants bearing wrinkled peas with those bearing smooth peas, producing 29,000 plants altogether. When he was done and he had run the numbers, he had exposed the gene.
This was the Holy Shit! moment that launched genetics’ Holy Shit! century
Mendel didn’t expose the physical gene, of course (that would come a century later), but the conceptual gene. And this conceptual gene, revealed in the tables and calculations of this math-friendly monk, seemed an agent of mathematical neatness. Mendel’s thousands of crossings showed that the traits he studied — smooth skin versus wrinkled, for instance, or purple flower versus white — appeared or disappeared in consistent ratios dictated by clear mathematical formulas. Inheritance appeared to work like algebra. Anything so math-friendly had to be driven by discrete integers.
It was beautiful work. Yet when Mendel first published his findings in 1866, just seven years after Charles Darwin’s On the Origin of Species, no one noticed. Starting in 1900, however, biologists rediscovering his work began to see that these units of heredity he’d discovered — dubbed genes in 1909 — filled a crucial gap in Darwin’s theory of evolution. This recognition was the Holy Shit! moment that launched genetics’ Holy Shit! century. It seemed to explain everything. And it saved Darwin.
Darwin had legitimised evolution by proposing for it a viable mechanism — natural selection, in which organisms with the most favourable traits survive and multiply at higher rates than do others. But he could not explain what created or altered traits.
Mendel could. Genes created traits, and both would spread through a population if a gene created a trait that survived selection.
That much was clear by 1935. Naturally, some kinks remained, but more math-friendly biologists soon straightened those out. This took most of the middle part of the 20th century. Biologists now call this decades-long project the modern evolutionary synthesis. And it was all about maths.
The first vital calculations were run in the 1930s, when Ronald Fisher, J B S Haldane and Sewall Wright, two Brits and an American working more or less separately, worked out how Mendel’s rather binary genetic model could create not just binary differences such as smooth versus wrinkled peas but the gradual evolutionary change of the sort that Darwin described. Fisher, Haldane and Wright, working the complicated maths of how multiple genes interacted through time in a large population, showed that significant evolutionary change often revealed itself as many small changes yielded a large effect, just as a series of small nested equations within a long algebra equation could.
The second kink was tougher. If organisms prospered by out-competing others, why did humans and some other animals help one another? This might seem a non-mathy problem. Yet in the 1960s, British biologist William Hamilton and American geneticist George Price, who was working in London at the time, solved it too with maths, devising formulas quantifying precisely how altruism could be selected for. Some animals act generously, they explained, because doing so can aid others, such as their children, parents, siblings, cousins, grandchildren, or tribal mates, who share or might share some of their genes. The closer the kin, the kinder the behaviour. Thus, as Haldane once allegedly quipped, ‘I would lay down my life for two brothers or eight cousins.’
Thus maths reconciled Mendel and Darwin and made modern genetics and evolutionary theory a coherent whole. Watson and Crick’s 1953 discovery of the structure of DNA simply iced the cake: now we knew the structure that performed the maths.
Finally, also in the 1960s, Hamilton and American George Williams upped the ante on the gene’s primacy. With fancy maths, they argued that we should view any organism, including any human, as merely a sort of courier for genes and their traits. This flipped the usual thinking. It made the gene vital and the organism expendable. Our genes did not exist for us. We existed for them. We served only to carry these chemical codes forward through time, like those messengers in old sword-and-sandal war movies who run non-stop for days to deliver data and then drop dead. A radical idea. Yet it merely extended the logic of kin selection, in which any gene-courier — say, a mom watching her children’s canoe overturn — would risk her life to let her kin carry forth her DNA.
This notion of the gene as the unit selected, and the organism as a kludged-up cart for carrying it through time, placed the gene smack at the centre of things. It granted the gene something like agency.
At first, not even many academics paid this any heed. This might be partly because people resist seeing themselves as donkey carts. Another reason was that neither Hamilton nor Williams were masterly communicators.
But 15 years after Hamilton and Williams kited this idea, it was embraced and polished into gleaming form by one of the best communicators science has ever produced: the biologist Richard Dawkins. In his magnificent book The Selfish Gene (1976), Dawkins gathered all the threads of the modern synthesis — Mendel, Fisher, Haldane, Wright, Watson, Crick, Hamilton, and Williams — into a single shimmering magic carpet.
These days, Dawkins makes the news so often for things like pointing out that a single college in Cambridge has won more Nobel Prizes than the entire Muslim world, that some might wonder how he ever became so celebrated. The Selfish Gene is how. To read The Selfish Gene is to be amazed, entertained, transported. For instance, when Dawkins describes how life might have begun — how a randomly generated strand of chemicals pulled from the ether could happen to become a ‘replicator’, a little machine that starts to build other strands like itself, and then generates organisms to carry it — he creates one of the most thrilling stretches of explanatory writing ever penned. It’s breathtaking.
Dawkins reveals the gene as not just the centre of the cell but the centre of all life, agency, and behaviour
Dawkins assembles genetics’ dry materials and abstract maths into a rich but orderly landscape through which he guides you with grace, charm, urbanity, and humour. He replicates in prose the process he describes. He gives agency to chemical chains, logic to confounding behaviour. He takes an impossibly complex idea and makes it almost impossible to misunderstand. He reveals the gene as not just the centre of the cell but the centre of all life, agency, and behaviour. By the time you’ve finished his book, or well before that, Dawkins has made of the tiny gene — this replicator, this strip of chemicals little more than an abstraction — a huge, relentlessly turning gearwheel of steel, its teeth driving smaller cogs to make all of life happen.
It’s a gorgeous story. Along with its beauty and other advantageous traits, it is amenable to maths and, at its core, wonderfully simple. It has inspired countless biologists and geneticists to plumb the gene’s wonders and do brilliant work. Unfortunately, say Wray, West-Eberhard and many others, the selfish-gene story is so focused on the gene’s singular role in natural selection that in an age when it’s ever more clear that evolution works in ways far more clever and complex than we realise, the selfish-gene model increasingly impoverishes both scientific and popular views of genetics and evolution. As both conceptual framework and metaphor, the selfish-gene has helped us see the gene as it revealed itself over the 20th century. But as a new age and new tools reveal a more complicated genome, the selfish-gene is blinding us.
For over two decades, Wray, West-Eberhard and other evolutionary theorists — such as Massimo Pigliucci, professor of philosophy at the City University of New York; Eva Jablonka, a geneticist and historian of science at Tel Aviv University, London; Stuart Kauffman, professor of biochemistry and mathematics at the University of Vermont; Stuart A Newman, professor of cell biology and anatomy at the New York Medical College; and the late Stephen Jay Gould, to name a few — have been calling for an ‘extended modern synthesis’ to replace the gene-centric view of evolution with something richer. They do so even though they agree with most of what Dawkins says a gene does. They agree, in essence, that the gene is a big cog, but would argue that the biggest cog doesn’t necessarily always drive the other cogs. In many cases, the other cogs drive the gene. The gene, in short, just happens to be the biggest, most obvious part of the trait-making inheritance and evolutionary machine. But not the driver.
Another way to put it: Mendel stumbled over the wrong chunk of gold.
Mendel ran experiments that happened to reveal strong single-gene dynamics whose effects — flower colour, skin texture — can seem far more significant than they really are. Many plant experiments since then, for instance, have shown that environmental factors such as temperature changes can spur gene-expression changes that alter a plant far more than Mendel’s gene variants do. As with grasshoppers, a new environment can quickly turn a plant into something almost unrecognisable from its original form. If Mendel had owned an RNA sequencing machine and was in the habit of tracking gene expression changes, he might have spotted these. But sequencers didn’t exist, so he crossed plants instead, and saw just one particularly obvious way that an organism can change.
The gene-centric view is thus ‘an artefact of history’, says Michael Eisen, an evolutionary biologist who researches fruit flies at the University of California, Berkeley. ‘It rose simply because it was easier to identify individual genes as something that shaped evolution. But that’s about opportunity and convenience rather than accuracy. People confuse the fact that we can more easily study it with the idea that it’s more important.’
The gene’s power to create traits, says Eisen, is just one of many evolutionary mechanisms. ‘Evolution is not even that simple. Anyone who’s worked on systems sees that natural selection takes advantage of the most bizarre aspects of biology. When something has so many parts, evolution will act on all of them.
‘It’s not that genes don’t sometimes drive evolutionary change. It’s that this mutational model — a gene changes, therefore the organism changes — is just one way to get the job done. Other ways may actually do more.’
Like what other ways? What significant and plausible evolutionary dynamics stand in tension with a single-gene-centred model? What gets obscured by the insistence that a ‘selfish gene’, a coherent, solitary replicator, is the irreducible and ever-present driver of evolution?
A shortlist of such dynamics would include some of the evolutionary dynamics being proposed by anthropologists, such as cultural transmission of knowledge and behaviour that allow social species ranging from bees to humans to adapt to changing environments without genetic alterations; and culture-gene evolution, a related idea, in which culture is not the ‘handmaiden’ of genes, but another source of transmissible adaptive information whose elements co-evolves with genes, each affecting the other.
Also in tension with the selfish-gene model are epigenetic changes suggested by recent research, such as methylation and other alterations to chemical wrappings around DNA, that can modulate DNA’s expression without changing its sequence. Such epigenetic changes may provide a way to pass heritable traits down through at least a few generations without changing any actual genes. To be sure, this research is still unproven as a significant evolutionary force. But while it is clearly important enough to pursue, many defenders of the selfish-gene model dismiss it out of hand.
Finally, the selfish-gene model is in tension with various ‘interesting evolutionary phenomena’, as Gregory Wray puts it in Evolution: The Extended Synthesis, ‘that are apparent only at the scale of hundreds or thousands of genes’ — a scale only made viewable during the past decade or so, as we’ve learnt to rapidly sequence entire genomes.
Of these genomic dynamics, perhaps the most challenging to the selfish-gene story are epistatic or gene-gene interactions. Epistasis refers to the fact that the presence of some genes (or their variants) can have profound and unpredictable influences on the activity and effects generated by other genes. To put it another way, a gene’s effect can vary wildly depending on which combination of other genes it finds itself with. (Think Jerry Garcia playing with different musical partners.)
Epistasis is hardly a new concept. In fact, geneticists have been arguing about its importance ever since R.A. Fisher and Sewall Wright bickered about it in the 1920s. Dawkins acknowledges a role for gene-gene interactions in The Selfish Gene, noting that ‘the effect of any one gene depends on interaction with many others.’ But research since then show that these interactions take place in non-linear, non-additive ways of a complexity impossible to understand at the time Dawkins wrote his book. Casey Greene and Jason Moore of Dartmouth, for instance, recently found that in some cases epistatic interactions seem to warp conventional gene-trait relationships so profoundly that they can often negate the gene as a trait’s reliable carrier.
Individual bees morph from worker to guard to scout by gene expression alone, depending on the needs of the hive
This is not merely a matter of one gene muffling or amplifying another, though both these things happen. And it’s not a matter of additive effects, such as four ‘tall’ genes making you taller than would two. Rather, these multi-gene epistatic interactions can create endless possible combinations of mutual influence in which any given gene’s contribution seems to rise less from its inherent trait-making power than from what company that gene finds itself keeping. To draw on P.Z. Myers’ apt analogy, epistasis means that single genes often carry little more inherent significance than individual playing cards do in poker. In a poker hand, the significance and effect of a two of hearts — its ‘trait’ — depend so heavily on the other cards you’re holding that it’s almost meaningless to say the card has any replicable power on its own. It’s replicable in that it’s a two of hearts every time it’s dealt. But it can deliver the same effect in subsequent generations only if it’s dealt not just into the exact same handful of cards, but into a round in which all the other players at the table also hold the same cards as before — and happen to bet, hold, and fold in exactly the same way. Not something to count on.
And a two of hearts is a far more coherent thing than is a gene. One of the peskiest problems of leaning too heavily on a gene-centric model these days is that the definition of the word ‘gene’ gets ever more various and slippery.
Even as a technical term, the word carries at least a half-dozen meanings, and more are added as science finds new tools for exploring the genome. This alone makes it either a poor candidate for a popular meme — or, if you value flexibility over exactitude, perhaps a perfect one, since its meaning can be defended or reshaped or expanded to suit the occasion. If you expand the meaning to be ‘the thing essential to all true heredity and selection’, you can then give the gene primary credit for any discovered or proposed evolutionary force in which the gene seems to be involved — and reject outright any proposed evolutionary force that doesn’t seem to involve genes.
But the gene’s definition is not just semantically vague. As geneticists explore the genome’s previously uncharted stretches, they’re finding that a lot of the work conventionally attributed to ‘genes’ (in the sense of consistent, reasonably well defined clusters of DNA) appears to be done instead by networks of genes and strange DNA elements that doubly defy the selfish-gene model.
These regulatory networks challenge the selfish-gene model first because they include DNA elements not conventionally defined as genes. More important, some researchers believe these networks challenge the selfish-gene model because they often seem to behave not like selfish entities balancing their separate agendas, in selfish-gene style, but like managerial teams regulating the behaviour of individual genes for the interest of the organism. The chromosome’s three-dimensional nature brings those regulatory chunks into contact with individual genes in highly unpredictable ways. With each gene ‘surrounded by an ocean’ of such regulatory elements, as molecular biophysicist Joe Dekker told WIRED, each gene ‘can touch and interact with a whole collection of them’. Yale geneticist Mark Gerstein found that the genes in these networks sometimes seem to get selected for even if they don’t have important effects on their own. In other cases they seem to have effects but be exempt from selection pressure.
These regulatory elements now appear to grossly outnumber the actual genes, possibly by as much as 50 to 1. As Yale geneticist Mark Gerstein politely notes, the complexity of these regulatory networks, along with their ad hoc management-team nature, raise the question of what’s being selected: individual genes, as the selfish-gene model proposes, or the management team, by some process still hidden amid all this complexity. Others, such as Cold Spring Harbor geneticist Thomas Gingeras, question outright whether the transcript (the marching orders a gene issues to begin gene expression) should replace the gene as the genome’s functional unit. These issues are not merely academic; resolving them could help solve mysteries about cancer and other diseases.
Such dynamics have emerged only in the last decade or so, as researchers have been able to examine the genome more closely. Yet even though we so far ‘have only a dim idea of how all this works’, as Gregory Wray wrote in 2010, it ‘is clear … that these kinds of assumption-violating exceptions are not rare.’
Wray’s language here is crucial: he’s not saying these findings refute the details of the gene-centric model. He’s saying they violate the model’s assumptions.
And this is the crux of this entire dispute: The point is not whether the findings of a genomic age or of anthropology refute the selfish-gene model, invalidate its theoretical details, or debunk the modern synthesis. Mostly they don’t. The selfish-gene model is roomy enough to host many of these findings. It has shown a uncanny ability to do so. But as time passes it does so ever more uncomfortably, for both host and guests. Some findings or ideas must be almost forced in. Others get prematurely locked out.
The selfish-gene model and metaphor can probably be stretched even more to account for some of these things. But in an age when assumption-violating ideas from genomic studies, anthropology, and other fields are flourishing, does the selfish gene story remain the best way to account for them? Does it make sense to attach these proliferating findings and ideas on to the selfish-gene story as appendices? Or is it time to find another story? It may be that the gene is always a player. But it is rarely the only player. And — may I speak metaphorically? — it may (or may not) be that the gene always behaves as if it were selfish. But that doesn’t mean it always gets its way.
One of the assumption-violating exceptions Wray refers to is gene expression’s breadth of power. In the social wasps that Mary Jane West-Eberhard has been studying in Panama since 1979, many of the most important distinctions among a colony’s individuals rise not from differences in their genomes, which vary little, but from the plasticity born of gene expression. This starts with the queen, who is genetically identical to her thousands of sisters yet whose gene expression makes her not only larger, but singles her out as the colony’s reproductive unit. Likewise with most honeybees. In social honeybees, the differences between workers, guards, and scouts all arise from gene expression, not gene sequence. Individual bees morph from one form to another — worker to guard to scout — by gene expression alone, depending on the needs of the hive.
As described above, the questionable coherence of genes seems to apply especially to gene regulation — as do epistatic networks that further undermine the gene’s primacy. So while it’s clear that DNA plays a key role in regulating gene expression, it is not clear that all these ‘regulatory genes’ are the selfish genes of the Dawkins model.
This is but one reason why West-Eberhard, among others, has been long trying to cure the ‘cyclic amnesia’ that she says has ignored 150 years of evidence that the gene’s centrality is overplayed. West-Eberhard is a particularly articulate advocate. Yet she’s frustrated at how little she’s been able to change things.
As a David to Dawkins’s Goliath, West-Eberhard faces distinct challenges. For starters, she’s a she while Dawkins is a he, which should not matter but does. And while Dawkins holds forth from Oxford, one of the most prestigious universities on earth, and deploys from London an entire foundation in his name, West-Eberhard studies and writes from a remote outpost in Central America. Dawkins commands locust-sized audiences any time he speaks and probably turns down enough speaking engagements to fill five calendars; West-Eberhard speaks mainly to insect-crazed colleagues at small conferences. Dawkins wrote a delicious 300-page book that has sold tens of millions of copies; West-Eberhard has written a bunch of fine obscure papers and an 800-page tome, Developmental Plasticity and Evolution (2003), which, though not without its sweet parts, is generally consumed as a meal of obligation.
She does have her pithy moments. There are times, she says, when ‘the gene does not lead. It follows.’
Massimo Piglucci and Gerd Muller use the same language in Evolution: The Extended Synthesis. By ‘the gene follows’, they mean that in complex organisms particularly, dynamics other than gene alterations, ranging from gene expression to complex gene regulation to developmental pathways formed by culture, can create heritable adaptations that either remain on their own or later become ‘fixed’ or locked in by genes.
One way in which the gene follows is through genetic assimilation — a clunky term for a graceful process. This can look Lamarckian, but it is not. It’s the development of a heritable change through flexible gene-expression responses that later get ‘fixed,’ or locked in, by a change in genotype. It takes a moment to explain. But let’s give it a run.
Genetic assimilation involves a three-step process.
First, an organism adapts to a changing environment by altering its gene expression to change its phenotype — its form or behaviour. Second, a gene emerges that locks in that phenotypic change. Finally, the gene spreads through the population.
For example, suppose you’re a predator. You live with others of your ilk in dense forest. Your kind hunts by stealth: you hide among trees, then jump out and snag your meat. You needn’t be fast, just sneaky and quick off the mark.
They didn’t inherit your speed in any Lamarckian way. Rather, like you, they simply developed it through gene expression driven by running so much
Then a big event — maybe a forest fire, or a plague that kills all your normal prey — forces you into a new environment. This new place is more open, which nixes your jump-and-grab tactic, but contains juicy little wild pigs that you can outrun if you sprint really hard. You start running down these critters. As you do, certain genes ramp up expression to build more muscle and fire the muscles more quickly. You get faster. You’re becoming a different animal.
You mate with another hunter. Your kids grow up to hunt with you. Since they hunt and practice hunting from early on, they too become fast — maybe faster than you, since they started younger. They didn’t inherit your speed in any Lamarckian way. Rather, like you, they simply developed it through gene expression driven by running so much. The same thing happens with their children: they run early, so they’re fast. Their speed is environmentally dependent. Your descendants keep this greater speed (greater, that is, than your ancestors’) for as long as they’re running down little pigs. The hunt makes them faster. But if they could get meals without sprinting, their speed would fade.
Now comes the second step: Several generations down the line, a beneficial mutation occurs in one of your descendants. Most mutations are neutral and many are bad. But this one’s good: It creates faster muscle fibres that let this descendant of yours — let’s call her Diana — easily run faster than her fastest siblings and cousins ever could. She flies.
Finally comes the third step: Diana’s children inherit the gene, as do some of theirs, and because their speed wows their mating prospects, Diana’s descendants mate early and often, bearing many kids. Thus this runner’s gene spreads through the generations until it becomes fixed in the population.
Now the thing is complete. An adaptive trait you originally developed through gene expression alone is made more permanent in your descendants by a new gene. Had the gene showed up back when you lived in the forest and speed didn’t mean anything, it would have given no advantage, and instead of being selected for, that speed gene would have disappeared or remained present but uncommon. But because hunting gave the gene value, the population took it in and spread it wide. The gene didn’t drive the train; it hopped aboard.
This isn’t the gene-centric world in which genotype creates phenotype. It’s a phenotype accommodating a new genotype by making it valuable.
Genetic assimilation was recognised as a possibility in the 1940s, but as Massimo Pigliucci and Courtney Murren put it, it was ‘attacked as of minor importance during the ‘hardening’ of the neo-Darwinian synthesis and … relegated to a secondary role for decades’. Interest has surged lately as gene expression becomes more apparent, and biologists are starting to spot the process in the field. No one proposes that genetic assimilation happens all the time or even commonly, or that it widely replaces conventional gene-driven evolution. But its existence suggests how gene expression’s fluidity can combine with conventional genetic dynamics to broaden evolution’s reach.
Gene Robinson, an entomologist who studies honeybees at the University of Illinois, says genetic assimilation could well have helped to create African honeybees, the ‘killer bee’ subspecies that is genetically distinct from the sweeter European honeybees that most beekeepers keep. Honeybee hives in certain parts of Africa, he says, were and are raided by predators more often than hives elsewhere, so their inhabitants had to react more sharply to attacks. This encouraged gene-expression changes that made the African bees respond more aggressively to threat. When new genes showed up that reinforced this aggression, those genes would have been selected for and spread through the population. This, Robinson says, is quite likely how African bees became genetically distinct from their European honeybee cousins. And they’d have been led there not by a gene, but by gene expression.
After several weeks of reading and talking to this phenotypic plasticity crowd, I phoned Richard Dawkins to see what he thought of all this. Did genes follow rather than lead? I asked him specifically about whether processes such as gene assimilation might lead instead. He said that genetic assimilation doesn’t really change anything, because since the gene ends up locking in the change and carrying it forward, it all comes back to the gene anyway.
‘This doesn’t modify the gene-centric model at all,’ he said. ‘The gene-centric model is all about the gene being the unit in the hierarchy of life that is selected. That remains the gene.’
‘He’s backfilling,’ said West-Eberhard. ‘He and others have long been arguing for the primacy of an individual gene that creates a trait that either survives or doesn’t.’
Yet West-Eberhard understands why many biologists stick to the gene-centric model. ‘It makes it easier to explain evolution,’ she says. ‘I’ve seen people who work in gene expression who understand all of this. But when they get asked about evolution, they go straight to Mendel. Because people understand it more easily.’ It’s easy to see why: even though life is a zillion bits of biology repeatedly rearranging themselves in a webwork of constantly modulated feedback loops, the selfish-gene model offers a step-by-step account as neat as a three-step flow chart. Gene, trait, phenotype, done.
In other words, the gene-centric model survives because simplicity is a hugely advantageous trait for an idea to possess. People will select a simple idea over a complex idea almost every time. This holds especially in a hostile environment, like, say, a sceptical crowd. For example, Sean B Carroll, professor of molecular biology and genetics at the University of Wisconsin, spends much of his time studying gene expression, but usually uses gene-centric explanations, because when talking to the public, he finds a simple story is a damned good thing to have.
Which drives West-Eberhard nuts.
‘Dawkins understands very well that gene expression is powerful,’ she says. ‘He sees things are more complex than a selfish gene. He could turn on its head the whole language.’
Yet Dawkins, and with him much of pop science, sticks to the selfish gene. The gene explains all. So far it has worked. The extended synthesis crowd has published scores of papers, quite a few books, and held meetings galore. They have changed the way many biologists think about evolution. But they have scarcely touched the public’s understanding. And they have not found a way to displace a meme so powerful as the selfish gene.
This meme, methinks, forms the true bone of contention and the true obstacle to progress. It’s one of the odd beauties of this whole mess that Dawkins himself coined the term meme, and did so in The Selfish Gene. He defined it as a big idea that competes for dominance in a tough environment — an idea that, like a catchy tune or a good joke, ‘propagates itself by leaping from brain to brain’. The selfish-gene meme has done just that. It has made of evolutionary theory a vehicle for its replication. The selfish gene has become a selfish meme.
If you’re West-Eberhard or of like mind, what are you to replace it with? The slave-ish gene? Not likely to leap from brain to brain. The co-operative gene? Dawkins himself considered this but rejected it and I agree that it lacks sufficient bling. And as West-Eberhard notes, any phrase with ‘gene’ in it still encourages a focus on single genes. And ‘evolution is not about single genes,’ she says. ‘It’s about genes working together.’
Perhaps better then to speak not of genes but the genome — all your genes together. And not the genome as a unitary actor, but the genome in conversation with itself, with other genomes, and with the outside environment. If grasshoppers becoming locusts, sweet bees becoming killers, and genetic assimilation are to be believed it’s those conversations that define the organism and drive the evolution of new traits and species. It’s not a selfish gene or a solitary genome. It’s a social genome.
What would Mendel think of that? Let’s play this out.
Mendel actually studied bees as a boy, and he studied them again for a couple years after he finished his pea-plant studies. In crossbreeding two species at the monastery, he accidentally created a strain of bees so vicious that he couldn’t work with them. If he’d had an RNA sequencer, he, like Gene Robinson, could have studied how much of the bees’ aggression rose from changes in the genetic code or how much rose from gene expression in response to the environment. If he had, the father of genetics might have seen right then that traits change and species evolve not just when genes change, when a creature and its genome and hive mates respond to an environment. He might have discovered not just genes, but genetic assimilation. Not the selfish gene, but the social genome.
Alas, no such equipment existed, and Mendel worked in a monastery in the middle of town. His vicious bees promised not a research opportunity but trouble. So he killed them. He would found genetics not through a complex story told by morphing bees, but through a simple tale told by one pea wrinkled and one pea smooth.
This is a revised version of the essay ‘Die, Selfish Gene, Die’. It replaced the original on 13 December 2013. Reader comments posted before that date were responding to the original version. A post at the author’s blog explains the revision and includes some other resources.